Today’s guest blog is written by Ken Doyle, a Science Writer with Promega. Reposted from the Promega Connections blog with permission.
There’s a certain group of people (including this blog post author) who derive no small amount of amusement from seeing stock photos of DNA and pointing out flaws in the structure. It’s even more amusing when these photos are used in marketing by life science companies. The most common flaw: the DNA molecule is a left-handed double helix.
What does that even mean? DNA, like many organic chemicals in biology, is a chiral molecule. That is, it can exist in two structural forms that are mirror images of each other but are not superimposable (enantiomers). Just like your left and right hands are mirror images of each other, the two DNA structures are left-handed and right-handed double helices. The DNA double helix is chiral, because its building blocks (nucleotides) are chiral.
It can be challenging, at first glance, to tell whether an image of DNA is left-handed or right-handed. Various helpful hints are available; however, the one that I’ve found easiest to remember is described in a blog post by Professor Emeritus Larry Moran at the University of Toronto:
Imagine that the double helix is a spiral staircase, and you’re walking down the staircase. If you’re turning to the right as you descend, the DNA structure is right-handed; if turning to the left, it’s left-handed. In the image shown earlier, the DNA molecule on the right is a right-handed double helix, while its mirror image is left-handed.
These fundamental concepts of DNA structure are summarized in an educational video from The Genetics Society.
B-DNA Rules the World
As is often the case in the real world, the story is a little more complex. DNA molecules can exist in several types of structures, both within cells and in vitro. The form common to all living organisms is a right-handed double helix, known as B-DNA. The two other common structures are Z-DNA (a left-handed double helix) and A-DNA (a right-handed double helix with other structural features that are distinct from B-DNA). Further, it’s important to note that DNA molecules such as those seen in stock images are idealized structures. In reality, Z-DNA is not a mirror image of B-DNA, and it has several other structural features, besides left-handedness, that distinguish it from B-DNA (1).
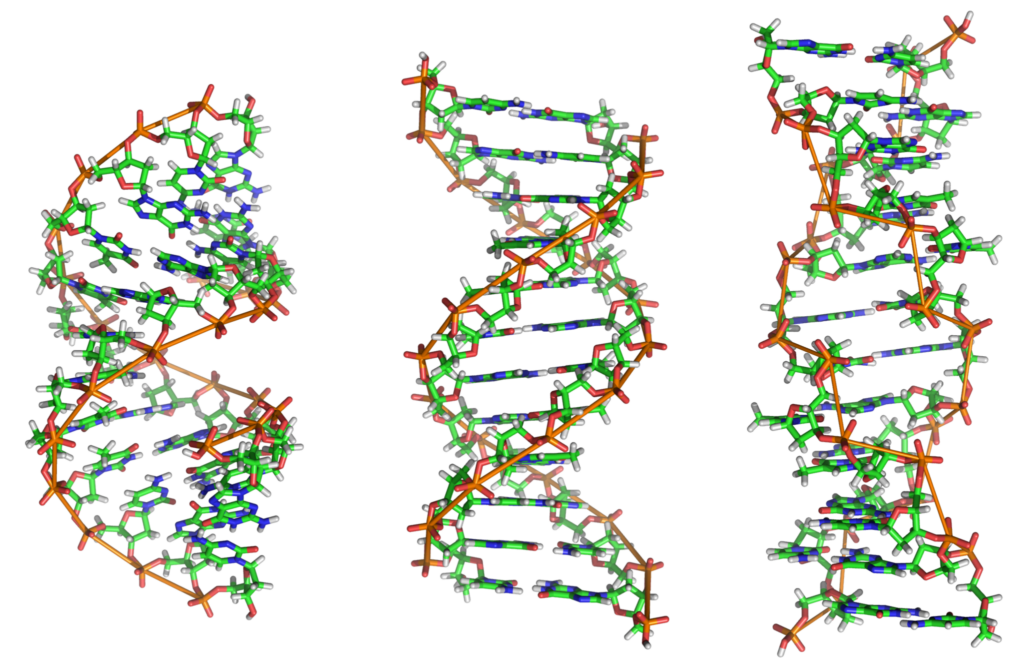
Why is B-DNA the overwhelmingly predominant form of DNA in all life forms? Several theories exist, but the one that has garnered the most attention is, literally, out of this world.
In 1962, two chemists in Cambridge, UK and Saarbrüchen, Germany published the results of research studying the effects of radiation on chemical molecules (2). It was known that the electrons in β-radiation produced by radioactive decay were chiral, in the sense that they could exist in two spin states that were mirror images. Interestingly, these electrons highly favored a left-handed spin state. Vester and Ulbricht investigated the interaction of this polarized radiation with chemical molecules to see if the radiation could induce chirality. Although they observed only a small effect, the Vester-Ulbricht hypothesis gained in popularity as an explanation for why nature favored one type of enantiomer when it came to biological molecules like sugars, amino acids—and DNA.
Over fifty years later, researchers at the University of Nebraska provided evidence that supported the Vester-Ulbricht hypothesis (3). They studied the chiral molecule bromocamphor and observed that polarized β-radiation preferentially dissociated one enantiomer of bromocamphor in the gas phase. That is, left-handed electrons preferentially destroyed left-handed bromocamphor enantiomers. Once again, the magnitude of this “chiral bias” effect was small, but it suggested the intriguing possibility that, in the distant past, polarized cosmic radiation could have selectively destroyed the precursors of left-handed DNA. A review of further research in this field suggests that, although the chiral bias observed is small, it can be persistent and have a major impact through billions of generations (4). Further, chiral bias also applies to RNA precursors, consistent with the “RNA world” hypothesis for the origin of life on Earth.
Why Z?
Although B-DNA is the dominant form of DNA in all organisms, Z-DNA does exist in vivo under certain conditions. In fact, there’s increasing evidence that it may play a variety of biological roles.
Z-DNA was discovered in 1970 when structural studies of poly d(I-C)·poly d(I-C), a synthetic DNA molecule, revealed an “unusual double-helical DNA” (5). Initially, Z-DNA was regarded merely as a biological curiosity. It could form under laboratory conditions that were far from physiological, such as high salt concentrations, or in repetitive GC sequences (6). However, further research showed that certain B-DNA structures could be converted transiently to Z-DNA in vitro. Even though B-DNA is the more stable form in solution, Z-DNA could be stabilized by chemical modifications and interaction with positively charged proteins (6).
A series of studies in the 1980s established biological roles for Z-DNA in autoimmune diseases and in transcriptionally active regions of genes (reviewed in 6). Subsequently, Z-DNA-binding domains were discovered in the human double-stranded RNA adenosine deaminase (ADAR1) enzyme, supporting the function of Z-DNA in actively transcribed regions of chromosomes (7). More recently, identification of Z-DNA binding domains in both host and viral proteins suggested its involvement in antiviral innate immunity (8).
Today, we know that Z-DNA is just one of several “alternative” DNA structures with biological functions. In addition to transcription, these alternative DNA structures are involved in DNA replication, DNA repair, genome stability and evolution (9, 10). The involvement of non-B-DNA in several diseases has spurred interest in developing drugs that specifically target ADAR1 and other proteins that bind non-B-DNA (9).
So, the next time you see a left-handed DNA stock image, consider this: instead of making an error, maybe the artist was speaking to a larger and more complex biological question!
WOULD YOU LIKE TO SEE MORE ARTICLES LIKE THIS? SUBSCRIBE TO THE ISHI BLOG BELOW!
SUBSCRIBE NOW!
References
- Ussery, D.W. (2001) DNA Structure: A-, B- and Z-DNA helix families. eLS DOI: 10.1038/npg.els.0003122
- Ulbricht, T.L.V. and Vester, F. (1962) Attempts to induce optical activity with polarized β-radiation. Tetrahedron 18, 629–637.
- Dreiling, J.M. and Gay, T.J. (2014) Chirally sensitive electron-induced molecular breakup and the Vester-Ulbricht hypothesis. Phys. Rev. Lett. 113, 118103.
- Globus, N. and Blandford, R.D. (2020) The chiral puzzle of life. Astrophys. J. Lett. 895, L11.
- Mitsui, Y. et al. (1970) Physical and enzymatic studies on poly d(I-C)·poly d(I-C), an unusual double-helical DNA. Nature 228, 1166–1169.
- Rich, A. and Zhang, S. (2003) Z-DNA: the long road to biological function. Nat. Rev. Genet. 4, 566–572.
- Herbert, A. et al. (1998) The Zα domain from human ADAR1 binds to the Z-DNA conformer of many different sequences. Nucleic Acids Res. 26(15), 3486–3493.
- Chiang, D.C. et al. (2021) The role of the Z-DNA binding domain in innate immunity and stress granules. Front. Immunol. 11, 625504.
- Wang, G. and Vasquez, K.M. (2022) Dynamic alternative DNA structures in biology and disease. Nat. Rev. Genet. DOI: 10.1038/s41576-022-00539-9
- Makova, K.D. and Weissensteiner, M.H. (2023) Noncanonical DNA structures are drivers of genome evolution. Trends Genet. 39(2), 109–124.